Previous Section || Go to Beginning || Next Section
Report of the Lens and Cataract Panel
PROGRAM OVERVIEW AND GOALS
Although most cells of the body undergo sequential processes of differentiation and aging, the lens cell is perhaps the simplest and most elegant system to study the molecular mechanisms involved in these two fundamental processes. In contrast to the cellular and molecular complexities present in most other tissues, the lens is a much simpler system, comprised of a single layer of epithelial cells that differentiate into fiber cells. Because of the very low turnover of proteins in the differentiated fiber cells, our lenses contain proteins that have been made during all ages of our life. The ease of obtaining lens epithelial and fiber cells, plus the relative molecular simplicity of the fully differentiated fiber cells, make the lens one of the best tissues to study the mechanisms that control the fundamental events of cell differentiation and aging.
Differentiation of epithelial cells to lens fiber cells involves the cessation of proliferation, remodeling of cellular architecture, and significant changes in the collection of genes that are expressed, resulting in a restriction of protein synthesis to the high-level expression of a few classes of proteins. In the last 5 years, it has become evident that differentiation of many cell types involves analogous changes in cellular behavior and a commitment to expression at high levels of cell type-specific genes. Hence, the lens is an excellent system to elucidate the control elements that dictate differentiation in many cell types.
During the later stages of fiber cell differentiation, protein synthesis is essentially terminated and proteolysis is limited. Fiber cells close to the lens center contain aged proteins, while cells close to the lens periphery contain newly synthesized proteins. By carefully peeling away sequential layers of cells, it is possible to obtain proteins of increasing age that date back to before the birth of the organism. Biochemists are just beginning to take advantage of this system to answer fundamental questions on how the aging process changes the nature of proteins.
Based solely on the contribution to understanding differentiation and aging, the lens is indeed an exciting system for study. Nonetheless, it is the transparent properties of the lens and its ability to focus light that present some of the most clinically relevant challenges to scientists involved in eye research.
For most people between the ages of 40 and 50, the ability of the lens to focus from distance to near (accommodate) has been compromised to the point where bifocals are required. The inability to adequately accommodate is known as presbyopia. It is the most common optical disorder of the eye. By understanding the changes in physical properties of the normal lens and its surrounding support structures as a function of age, it may be possible to develop treatments for presbyopia that delay or prevent its onset.
By far, the most serious problem associated with the lens is its loss of transparency. This condition, known as cataract, is the leading cause of blindness in the world. The disorder sometimes occurs in children, but most frequently occurs in adults age 50 and older. To date, there is no universally accepted pharmacological agent to either inhibit or reverse the progression of lens opacity. The only treatment is surgical removal of the lens, followed by implantation of an artificial lens at the time of surgery or the subsequent use of corrective lenses. The following statistics concerning cataract attest to its economic and public health importance:
- Cataract is the leading cause of blindness worldwide, accounting for about 42 percent of all blindness, in spite of the availability of an effective surgical treatment.
- With increasing life expectancy, the number of cases of blindness from this disorder may double by the year 2010.
- Among Medicare beneficiaries, cataract is the most common condition for which eyecare services are sought, accounting for 43 percent of visits to ophthalmologists and optometrists combined.
- In the United States, cataract surgery is the most frequently performed surgical procedure among 30 million Medicare beneficiaries. Approximately 1.35 million cataract operations are performed annually at an estimated cost of $3.5 billion.
Cataract is an immense medical problem. An eventual cure for cataract strongly depends on increased understanding of the basic molecular processes occurring in the normal and cataractous lens. The objectives listed in this report have been selected based on the assumption that not only are the basic processes of differentiation and aging important reasons for studying the lens, they also provide the framework for learning more about mechanisms involved in presbyopia and cataract. A fundamental understanding of the molecular mechanisms responsible for these two ocular disorders will bring researchers closer to discovering ways to prevent these diseases and developing effective drugs to treat them.
In Fiscal Year 1997, the National Eye Institute (NEI) funded 120 extramural research projects in the Lens and Cataract Program at a total cost of $25,368,000.
Following are the goals of the Lens and Cataract Program for the next 5 years.
- Understand the physiological basis of lens transparency on the cellular and molecular levels.
- Determine the causes and mechanisms of cataract formation.
- Characterize the controls of lens cell division and differentiation and their roles in the formation of posterior subcapsular and secondary cataracts.
- Understand lens development and the diseases associated with defects in this process.
ASSESSMENT OF PROGRESS
The discovery thatα-crystallins have molecular chaperone properties. The discovery that α-crystallins, a major component of lens fiber and epithelial cells, prevent denaturation and aggregation of proteins in vitro was unexpected. This novel finding suggested a particularly significant role for this important class of proteins, that of a molecular chaperone. Chaperones are proteins that affect protein-protein interactions by stabilizing protein conformations and preventing nonspecific protein aggregation in the face of heat denaturation or other environmental stresses. Whether the molecular chaperone-like properties of the α-crystallins play a key role in the prevention of protein denaturation and nonspecific aggregation during the aging of the human lens remains to be determined. However, recent findings with knockout mice lacking α-crystallins support the idea that a functional α-crystallin is critical for preventing opacification. Mice in which αA crystallin is missing develop opacities by the age of 7 weeks. Knocking out both αA and αB crystallins in mice results in even more severe cataracts. In vitro studies have shown that the molecular chaperone properties of the α-crystallins decrease during aging of the lens perhaps, as studies suggest, as a consequence of environmental insults (e.g., oxidizing agents, ultraviolet [UV] light, glycation) to the human lens. Further support for α-crystallin as a chaperone comes from studies of the central nervous system; αBcrystallin expression is greatly enhanced in neurons of patients with neurodegenerative diseases such as Alexander's disease and Alzheimer's disease, where αB crystallin colocalizes with aggregates of aberrant proteins. These findings are consistent with an increase in α-crystallin expression to prevent aggregation in cells that are stressed.
Progress in characterizing changes to lens proteins during human lens aging and cataract. A great deal of progress has been made in characterizing structural changes that occur to lens proteins during the normal aging process. Identifying sites where posttranslational modifications occur has been aided by mass spectroscopy. Spectroscopic analysis and two-dimensional gel electrophoresis have permitted testing of the hypothesis that specific posttranslational modifications to lens proteins lead to cataract formation. Surprisingly, although many modifications have been identified, none of these have been specifically associated with age-related cataract. Posttranslational modifications of lens proteins seem to be a function of normal aging rather than cataractogenesis. However, post- translational protein modifications of α-crystallin may be significant because the physical and chemical properties of the α-crystallins are known to change during aging.
The demonstration that the retinoblastoma protein has a central role in the lens cell cycle. One goal of understanding cell division in the lens is to identify targets for the development of drug therapies to inhibit the inappropriate cell division that causes posterior secondary cataract following removal of cataract lenses. Advances in this area have centered around the discovery that the retinoblastoma protein pRb is the central gatekeeper that prevents lens fiber cells from entering into the cell cycle and, hence, from proliferating. pRb is a nuclear protein that binds to members of the E2F family of transcription factors, blocking expression of E2F-regulated genes. Not only is pRb involved in cell cycle withdrawal, it also plays a key role in preventing apoptosis or programmed cell death. Mice with homozygous deletions of the Rb gene have provided definitive evidence that inactivation of pRb permits S phase entry and apoptosis in lens fiber cells. Apoptosis in Rb null mice is suppressed when p53, a proapoptotic gene, is deleted along with Rb, suggesting that p53 monitors the cell cycle and prevents tumorigenic proliferation of lens cells.
Progress in defining the role of growth factors in the development and health of the lens. Growth factors are involved in all stages of lens development, and their relevance to maintaining a healthy lens has been firmly established. Over the last 5 years, much has been learned about how growth factors signal lens differentiation, regulate the cell cycle, and impact on lens transparency. Experiments using transgenic mice and lens explants indicate that members of the fibroblast growth factor (FGF) family are prime candidates for the retina-derived inducers of fiber cell differentiation during lens development and the molecules responsible for maintaining the balance between differentiation and division in the mature lens. Other studies using cultured lens epithelial cells suggest that insulin or insulin-related growth factors (IGFs) act synergistically with FGFs to induce fiber cell differentiation. Studies in transgenic mice point to a role for platelet-derived growth factor (PDGF) in stimulating epithelial cell proliferation in the mature lens. Lens epithelial cells express receptors for PDGF, whereas adjacent cells in the anterior chamber of the eye synthesize PDGF. Lens epithelial cells exposed to transforming growth factor-β (TGF-β) are induced to assume a spindle shape and synthesize connective tissue proteins, such as collagen type I and α-smooth muscle actin, all characteristics of posterior subcapsular cataracts. Since latent TGF-β is present in the normal eye and can be activated by trauma or surgery, TGF-β may be a significant factor in posterior subcapsular cataracts.
Expanding the understanding of lens development. Identifying mutations in the Pax-6 gene as being responsible for causing aniridia, a congenital malformation of the eye, was a major breakthrough not only in understanding this disease but also in understanding the developmental processes controlling eye development. This was the first gene to be shown by genetic function to be essential for normal vertebrate eye development. The insight was especially profound because of the nature of the gene: Pax-6 is a member of the homeobox transcription factor family, which is at the heart of the hierarchy of genes controlling formation of the body plan in Drosophila. The significance of Pax-6 as a key regulator of eye formation in general, and of determination and differentiation of the lens in particular, has been substantiated in a number of experimental systems. The small-eye mutation sey in mice maps to Pax-6. In addition, ectopic expression in Drosophila embryos of the Drosophila Pax-6 gene ey caused formation of extra eyes at a number of sites on these animals. These experiments led researchers to conclude that Pax-6 is a master gene that controls the expression of downstream genes during eye development. This has led to a search for other developmentally regulated genes and promoter elements that are recognized by Pax-6. Recent work suggests that there are a number of regulatory genes, most of which encode transcription factors, that are also essential for normal eye development. These include the homeobox genes Optx-2 and Six-3, and the transcriptional regulator genes Sox-2 and Rx-1.
The identification and characterization of gap junction proteins.Gap junctions contain intercellular channels that provide an aqueous pathway between adjacent cells, allowing them to share ions and small molecules. Because the lens is avascular it has been hypothesized that gap junctions between lens cells play a crucial role in intercellular metabolic support essential for lens survival. Through molecular biological and immunochemical approaches, three proteins (called connexins) that form lens gap junctions have been identified, cloned, and sequenced. Lens epithelial cells are coupled by channels formed from connexin 43. Mammalian fiber cell gap junctions are formed from connexin 46 and connexin 50. The importance of gap junctions in maintaining transparency has recently been demonstrated with the use of knockout technology. Mutant mice in which the gene for the fiber-specific connexin (connexin 46) has been deleted developed nuclear opacities within 3 weeks of birth. The association of these nuclear cataracts with proteolysis of crystallins underscores the significance of gap junction to the overall homeostasis of the lens.
PROGRAM OBJECTIVES
- The objectives for the Lens and Cataract Program over the next 5 years include both laboratory and clinical research.
- Determine if there are novel markers that differentiate the normal aging process from the diseased (cataractous) state.
- Definitively test hypotheses of cataract.
- Map, identify, and characterize genes which, when mutated, cause congenital or age-related cataract; determine if there are genetic factors that interact with environmental factors to confer susceptibility to age-related cataract.
- Identify genes and pathways that control eye development, especially those critical for lens induction, cell fate determination, and cell differentiation.
- Define the contributions of crystallins to normal lens function.
- Characterize the control of the cell cycle in lens epithelial cells by identifying cell cycle regulators, growth factors, receptors, and signal transduction pathways.
- Characterize, at the molecular level, the ion channels, transporters, and gap junction proteins needed to maintain lens homeostasis; determine what roles perturbations in these systems play in cataract formation.
- Define the mechanisms that regulate the cellular and subcellular architecture of the lens, with special emphasis on the contribution of minor constituents and their progressive modification during aging and opacification.
- Understand the basis of lens accommodation and presbyopia at the molecular and mechanistic levels.
The needs and opportunities related to each of these objectives and the strategies for accomplishing them will now be considered.
Objective 1: Determine if there are novel markers that differentiate the normal aging process from the diseased (cataractous) state.
Research Needs and Opportunities
The human age-related cataractous lens is characterized by a lens opacity that is significant enough to decrease the visual acuity of the patient. Although acuity loss is the clinical manifestation of the disorder, the changes in the lens are likely the result of a series of biochemical and biophysical events, occurring over many years, that ultimately lead to opacification. The overall objective is to identify markers in the human lens that will clearly differentiate the processes of aging from cataractogenesis.
Identification and possible quantitation of such markers would significantly aid in understanding the mechanisms that lead to cataractogenesis. This information will help in developing pharmacological agents that can inhibit lens opacification and assist in the early diagnosis of patients who may benefit most from cataract therapy. In addition, identifying novel markers for cataract will permit the development of animal model systems that can approximate the biochemical and biophysical events occurring in the human lens. These animal model systems will be valuable in the preliminary screening of possible anticataract drugs. Following are general categories of possible markers:
- Biochemical markers of the lens epithelium, especially those involving changes in gene expression or mutations in specific genes.
- Lens protein alterations, especially those involving posttranslational modifications.
- Morphological and optical markers that can be visualized by noninvasive techniques.
Strategic Research Questions
Relative to the normal aging lens, are there differences in DNA coding sequences that may quantitatively or qualitatively affect specific enzymes or structural proteins that are consistently associated with opacification of the human lens? Although there are very small amounts of nucleic acid present in the lens epithelium and lens fiber cells, polymerase chain reaction (PCR) technology has now made it possible to amplify specific DNA or RNA sequences. Primers corresponding to important nucleic acid sequences could be used to amplify and probe for sequences that may be predictive for cataract.
Relative to the normal aging lens, are there qualitative or quantitative changes in posttranslational modifications of lens crystallins or membrane proteins during opacification of the human lens? Recent advances in mass spectrometry and high-pressure liquid chromatography (HPLC) have now made it possible to identify all major posttranslational modifications of proteins from the human cataractous lens. Emphasis should be placed on the use of mass spectral instrumentation connected online with HPLC. After identifying posttranslational modifications, HPLC can be used to quantitate the relative amounts of modification in cataractous versus normal lenses.
Relative to the normal aging lens, are there morphological changes in lens epithelial or lens fiber cells that occur before the onset of opacification of the lens? Advances in confocal microscopy and nuclear magnetic resonance (NMR) imaging have now made it possible to visualize changes in lens morphology and biochemistry before the onset of opacification. These techniques can be used to monitor various parameters of the lens in a noninvasive manner, make earlier diagnoses of cataract, and identify novel markers that characterize the precataractous human lens.
What optical markers of the eye reflect early cataractous changes, and can such optical signatures be correlated with suspected risk factors for cataract? Until recently, it has been difficult to measure the optical properties of the eye in vivo. The marked cost reduction of the high-performance charged coupled device (CCD) camera, together with development of the appropriate software, now make clinical measurement of optical aberrations fast, easy, and noninvasive. These advances provide the opportunity to quantify optical changes in the normal eye indicative of cataract formation and monitor cataract progression.
Objective 2: Definitively test hypotheses of cataract.
Research Needs and Opportunities
Age-related cataracts are the most important types of cataract from a public health perspective. Different types of cataract, classified primarily on whether they are nuclear or cortical, have characteristic morphologies, yet there is a tendency to generalize about cataracts and their causes.
Very little is known about what, in conjunction with age, initiates the formation of cataracts. This is, in part, because the cataractous lens is the end result of processes that occur over many years, even decades. The major initiating factors that have been proposed as a result of epidemiological studies are listed in the following figure. There is no compelling evidence that any of these factors are the primary cause of cataracts. In fact, the initiation of cataracts is probably the result of varying combinations of these factors, which interact in some complex manner.
The modes by which initiating factors affect the lens will be either direct, as in the case of UV exposure, or indirect, by altering levels of metabolites, growth factors, oxidants, antioxidants, autoantibodies, and so forth. Many investigators have suggested that the initial site of action for these factors is the lens epithelia, where altered epithelium function could affect underlying fiber cells. However, it is unclear if the initial target is the epithelia, the fiber cells, or the surrounding tissues, which secondarily impact the lens.
The major hypotheses proposed to explain age-related cataracts involve either a change in the protein-protein interactions leading to protein aggregation or membrane damage. Processes in the lens thought to affect one or both of these include: oxidative stress; posttranslational modification of crystallins; and, more recently, the loss of chaperone-like activity of α-crystallin. These have been considered key mechanisms leading to age-related cataract. A role for oxidative stress in age-related cataracts has been proposed, but the origin of the stress and the manifestation or relevance to any cataract have not been definitely demonstrated. The major posttranslational modifications of lens proteins seem to be a function of normal aging rather than cataractogenesis. A clear causative association between posttranslational modifications of lens proteins and age-related cataract has not been demonstrated.
DCataract formation paradigm
Each of the processes in the lens affected by the initiating factors (see figure above) has been shown to be related to cataract formation under special conditions or in special model systems. In human lenses, while some of these processes may be critical, there will likely be complex interconnections among them, generating a cascade of events that ultimately lead to cataract. Understanding the interrelationships between these processes will be imperative to elucidating mechanisms involved in cataract formation.
Strategic Research Questions
How can initiating factors be definitively identified? The late onset and likely multifactorial nature of age-related cataracts confounds the identification of initiating factors. The challenge to the scientific community is to design meaningful experiments that test causal agents identified in epidemiological studies. The lack of a well-defined animal model of age-related cataracts has hindered the implementation of such studies.
Do researchers have enough knowledge of the normal human lens to determine the relevant processes in cataracts? Baseline data on normal lens physiology and how it is affected as a result of the aging process are lacking. Large gaps in understanding how the lens maintains its homeostasis exist. Questions relating to cell division and differentiation, transport systems, and maintenance of protein structure are key to enhancing the understanding of normal lens physiology. How these processes change as a function of aging will provide clues as to their relevance to cataract formation.
To understand cataract, do researchers need to focus on tissues surrounding the lens? The initial site of damage in cataractogenesis is unknown. Theories of cataractogenesis postulate a role for agents originating in tissues external to the lens in the etiology of cataracts. The validity of these theories needs to be substantiated by demonstrating an ocular source for these agents. Conversely, the role of growth factors in maintaining the health of the lens is now becoming more apparent. These studies suggest the need to investigate the tissues that produce and secrete growth factors and how the lens utilizes growth factors in pursuing possible mechanisms of cataractogenesis.
What are the major contributing factors to oxidative stress, increased oxidants or decreased antioxidants, or both? A role for oxidative stress in development of age-related cataracts has been accepted. Strategies to determine how oxidative stress is manifested need to be devised to establish its relevance to cataract.
What is the functional relationship between the epithelial cells and fiber cells in normal lenses? Do epithelial cells accumulate altered DNA and subsequently differentiate into defective fiber cells? Many investigators have suggested that the initial site of damage is the lens epithelium, where altered epithelium function could affect underlying fiber cells. Identifying testable mechanisms depends on developing an understanding of the basic functional relationship between lens epithelial and fiber cells.
Objective 3: Map, identify, and characterize genes which, when mutated, cause congenital or age-related cataract; determine if there are genetic factors that interact with environmental factors to confer susceptibility to age-related cataract.
Research Needs and Opportunities
Age-related cataract is a complex disease that appears to be influenced by numerous environmental factors including, but not limited to, diet, UVB radiation, and smoking. While many epidemiological studies have suggested an association between these risk factors and cataract, very little is known concerning the identity of genes that either alone or together with environmental factors may confer susceptibility to cataract. As a start, results from the Beaver Dam Eye Study suggest that a single major gene defect may account for a sizable portion of both nuclear and cortical cataracts in this population.
Congenital cataract is less common than age-related cataract, but it is still responsible for 10 percent to 30 percent of all cases of blindness in children. While treatable, albeit not easily, congenital cataract is associated with a high rate of blindness because of a range of related clinical problems, including amblyopia, glaucoma, and strabismus. To date, nine distinct loci for autosomal dominant cataract have been mapped. The most common inheritance of congenital cataract in humans occurs through autosomal dominant transmission mapping of autosomal-dominant families has been important because it has demonstrated the presence of genetic and allelic heterogeneity allelic heterogeneity has been suggested by the close linkage of several clinically distinct phenotypes, including the Volkmann and the posterior polar cataract (1p36), the Coppock-like and the polymorphic lamellar cataract (2q33-36), and the Marner and posterior polar cataract (16q22.1). In contrast, genetic heterogeneity of autosomal-dominant cataract families with similar phenotypes has been demonstrated through gene mapping of similar phenotypes to separate loci. These include Cerulean cataract (17q24 and 22q) and anterior polar cataract (17p and a balanced reciprocal 2;14 chromosome translocation). Finally, two distinctly different inherited cataracts, zonular sutural cataract and zonular pulverulent cataract, have been mapped to 17q11-12 and 1q21-25, respectively.
To circumvent barriers of direct linkage studies in humans, mouse models have also been used to identify genes responsible for cataract development. Approximately 40 mouse mutants with cataract have been reported. Mutants that have had gene mutations identified include Philly (βB2 crystallin), Cat2 (γE crystallin), Cat Fraser (MIP), K289 (Pax-2), sey (Pax-6), No1 (connexin 50), and To3 (Lim2). The Cat Fraser mutant has been shown to have a splicing defect. The K289 mutant has a 1 bp insertion in the Pax-2 gene, which leads to a frameshift mutation. This mutation was identical to a mutation in a human family with renal-coloboma syndrome, and accentuates the importance of studying the mouse as model for human disease.
During the last 5 years, exciting discoveries have been made concerning the possible involvement of genetic factors in human age-related and congenital cataracts. With the development of sophisticated molecular biological approaches, it is now possible to directly address the hypothesis that defects in specific genes may be at least partially responsible for opacification of the human lens.
Strategic Research Questions
Do genetic mutations contribute to age-related or congenital cataract in humans and, if so, what are the identities of these genes? Linkage analysis for congenital forms of cataracts that are inherited as Mendelian-dominant or -recessive traits is routine. Newly developed methodology to study the genetics of complex diseases increases the likelihood that genetic loci associated with cataract can be directly identified.
Besides the Beaver Dam Eye Study, do other population-based studies also suggest the presence of genes that predispose individuals to cataract and, if so, do certain genes confer susceptibility to certain types of cataracts? What are the identities of these genes? Population-based studies are needed to determine if there is a genetic susceptibility to age-related cataract. If genetics is identified as a factor, studies to map these susceptibility genes need to be implemented.
If genetic mutations play a role in age-related or congenital cataracts, do they confer susceptibility alone, or must they act in the presence of certain environmental insults? With the identification of susceptibility genes, genetic epidemiology studies must be designed to address how and to what degree environmental insults such as UV irradiation, hormones, and nutrition contribute to the etiology of cataracts.
In animal and human cataract systems already partially characterized, what are the exact number of genes and what are the exact nucleotide changes occurring in the genes that are responsible for cataract formation? How do these mutations impair lens function? Complete genotypic and phenotypic characterization of these systems would accelerate genetic mapping studies of congenital and age-related cataracts by providing strong candidate genes.
Does further genetic mapping of mouse and human cataract systems suggest that the same genes may confer susceptibility to cataract in both systems? The use of mouse models has been a productive strategy of identifying candidate genes for human diseases. Continuation of this approach is needed. Studies to characterize the approximately 40 mouse mutants with cataract thus far identified need to be implemented and, once identified, the relevancy of these genes to human disease needs to be ascertained in genetic mapping studies.
Objective 4: Identify genes and pathways that control eye development, especially those critical for lens induction, cell fate determination, and cell differentiation.
Research Needs and Opportunities
Late in the last century, it was observed that the lens formed as a result of the interaction between embryonic ectoderm and the developing retina. This led to a series of classical experiments that suggested that lens formation was a simple process involving inductive signals sent from the presumptive retina, the optic vesicle, to the ectoderm. A number of experiments supported the idea that these signals were sufficient to stimulate lens determination in ectodermal tissue. With the discovery that the genetic disease aniridia is the result of mutations in a key regulatory gene, Pax-6, lens biologists using molecular techniques and markers have taken a fresh look at early developmental events. Recent studies now suggest that ectoderm acquires a lens bias in a regionalized pattern before the formation of the optic vesicle, and that this lens bias is associated with expression of a lens homeobox gene Pax-6. By the time of contact between the optic vesicle and ectoderm, regions of the ectoderm have already been committed to forming lens. These findings suggest that lens formation is a complex process requiring a number of tissue interactions and the hierarchical expression of a number of genes encoding transcription factors including Sox-2, Rx-1, and the homeobox genes Optx-2 and Pax-6.
These findings have for the first time allowed investigators to hypothesize a conceptual framework for lens determination. However, the interactions among these genes are not understood well enough to know how they coordinate lens formation, and there are likely to be many genes critical for this process that remain to be identified. Many of the identified genes are known to act as transcriptional regulators. While crystallins have been identified as targets of Pax-6 and other transcriptional regulators during lens differentiation, targets of these genes at the earlier stage of lens determination have yet to be defined. Far less is known about the signal transduction pathways that control lens induction and differentiation, though the technology and systems alluded to above can be applied to these questions.
Studies of lens development will benefit from approaches that integrate molecular genetics and embryology by allowing investigators to characterize cell fate genes by the timing of their expression and their place in the developmental hierarchy. As these developmental processes become defined, candidate genes for congenital cataract as well as other congenital diseases affecting the anterior segment will likely be identified. This will allow geneticists to select candidate genes based on their relevance to the developmental characteristics of the syndrome being mapped.
Strategic Research Questions
What are the key transcriptional regulators that control lens determination and differentiation? What is the hierarchical relationship among them and what is their mechanism of action? The identification of Pax-6 as a key regulator in lens determination has reshaped researchers' concepts of lens development. However, the total picture is just emerging, and as genes are discovered, gaps in knowledge will be filled in. Defining interactions among the genes and determining how they coordinate lens formation are critical to completing the picture.
How does the developing lens act to coordinate development of adjacent eye tissues? From studies of Pax-6 it is clear the lens plays a critical role in eye formation. The identification of additional lens- expressed genes and a demonstration of their role in eye development will provide a starting point to ask questions related to the role of the lens in overall eye development.
What are the genetic interactions, as defined by gain of function and loss of function experiments, that define the hierarchy of genes controlling lens determination? Experimental strategies that manipulate gene expression in model systems, either positively or negatively, should be highly useful in defining the gene interactions and determining hierarchical relationships in lens determination.
Do particular gene activities like those of Pax-6 control biologically defined phenomena such as lens specification and differentiation? Functional analysis is needed to identify specific roles for gene products of critical regulatory genes. This will require strategies that integrate classical embryological techniques with genetics and molecular biology.
What are the signals from tissues surrounding the developing lens? What are the receptors and pathways within the lens ectoderm that control its determination? Little is known about the signals and signal transduction pathways that control lens induction and differentiation. With technology advancing at a rapid rate, researchers can now approach these issues.
Objective 5: Define the contributions of crystallins to normal lens function.
Research Needs and Opportunities
The α-, β-, and γ-crystallins make up the bulk of the lens mass and are responsible for the larger refractive index of the lens. The α-crystallins, comprised of two highly related chains (αA and αB), account for approximately 40 percent of lens dry weight, while the families of β- and γ-crystallins, comprised of a least nine related proteins, account for almost all of the remaining dry weight of the lens. The relative contributions of each of the α, β, and γ proteins to the total refractile properties of the lens are unknown. In addition, nothing is known concerning the importance of each of the β and γ species in maintaining normal lens transparency, especially under conditions of metabolic stress. The roles of the α-, β-, and γ-crystallins in the possible control of molecular processes that occur during differentiation of the lens epithelial cells also remain undefined.
Recent findings indicate that α-crystallins possess molecular chaperone properties that enable them to inhibit nonspecific protein aggregation. This discovery has demonstrated a physiological role as well as a structural role for α-crystallin. In addition, significant amounts of αB crystallin have been found in other tissues besides the lens, including the retina, the heart, and the brain. Abnormally large amounts of αB crystallin are also found in some human neurological disorders. Both these observations suggest the intriguing possibility that the αB crystallin may also play physiological roles in other tissues.
This discovery of chaperone activity has provided the impetus for conducting further studies to characterize the structural and biochemical properties of this physiologically important process. The ability to perform structure and function studies has been aided by the techniques of molecular biology. The production of large amounts of unmodified crystallins is now possible. Genetic manipulations in mice have permitted the development of animal lines that either lack or overexpress specific α-, β-, or γ-crystallin polypeptides. Recent refinements in the use of cryoelectron microscopy and NMR spectroscopy now make the structural elucidation of large proteins that comprise macromolecular aggregates like α-crystallin feasible.
Strategic Research Questions
What are the molecular structures of the α-crystallins, and what parts of these structures are important in the molecular chaperone properties of the molecules? Technology such as cryoelectron microscopy and NMR spectroscopy now make it possible to address crystallin structure. New labeling techniques allow the spectroscopic identification of specific domains within molecular structures. The information gained from this technology is a first step in defining functional subdomains.
What are the preferred target proteins in the lens for chaperone function? Identifying target proteins will define the physiological role for chaperone function and serve to identify which lens proteins may play a role in cataract formation.
Are some forms of human cataract a direct result of the failure of α-crystallin to act as a molecular chaperone? Strategies such as analysis of loss of function mutants in model systems can be used to provide clues as to the role of this protein in maintaining transparency. From results in these systems, genetic mapping strategies and biochemical analysis can be pursued in humans.
Is the function of αB crystallin in the lens the same as outside the lens, and is this function related to abnormally high levels of expression in certain human disease states? Tissues outside of the lens can potentially reveal new insights into the function of α-crystallin in the lens. Much can be learned from pursuing such studies, particularly in regard to the stress-related role of α-crystallin in the disease process.
What possible roles do the α-, β-, and γ-crystallins play in lens differentiation? The roles of the α-, β-, and γ-crystallins in controlling molecular processes that occur during differentiation of the lens epithelial cells is an area that needs to be investigated.
What are the contributions of each of the β- and γ-crystallins to the transparent and refractile properties of the lens? Even though the crystallins make up the bulk of the lens mass and are responsible for the larger refractive index of the lens, little is known about the relative contributions of each of the α, β, and γ proteins to the total refractile properties of the lens. Nothing is known about the role of each of the β and γ species in maintaining normal lens transparency, especially under conditions of metabolic stress. Strategies to address these questions need to be developed.
Do any of the β- or γ-crystallins have functional roles in tissues other than the lens? Inherent in the concept of gene sharing in regard to the crystallins is the question of whether crystallins have functions in tissues outside of the lens.
Objective 6: Characterize the control of the cell cycle in lens epithelial cells by identifying cell cycle regulators, growth factors, receptors, and signal transduction pathways.
Research Needs and Opportunities
A substantial proportion of people who undergo cataract surgery develop secondary cataracts, a complication that increases the cost of cataract treatment because a second surgical procedure is required. Research has shown that secondary cataract formation is the result of a normal response of the residual lens epithelial cells that often remain after surgery. In the normal adult lens, cell division and differentiation are precisely regulated. After cataract surgery, however, the residual cells appear to be relieved of these controls and begin dividing in an attempt to form a new lens. If this process interferes with vision, laser treatment is required. It is essential to understand the regulation of proliferation and differentiation of these cells in order to devise new cost-effective preventive strategies.
Growth of the lens is regulated by controlled proliferation of lens epithelial cells and precisely localized induction of lens fiber cell differentiation. Lens epithelial cell proliferation appears to be regulated in part by synthesis of PDGF in the anterior chamber of the eye and in part by FGF stimulation near the lens equator. Differentiation into fiber cells appears to be induced by exposure to elevated levels of FGF, which may be acting in concert with IGFs. Signaling through specific integrins may also play a role in this process.
In contrast to the progress that has been made in identifying the extracellular factors that regulate lens growth and differentiation, very little is known about the specific signal transduction mechanisms they activate. While it is clear that PDGF, different members of the FGF family, and IGFs all act through receptors that have tyrosine kinase activity, the signaling pathways must be distinct in each case. Defining these differences will be crucial to understanding differentiation and cell cycle regulation in the lens. Moreover, since numerous pharmacological agents are available that target signal transduction pathways, information about the relevant signal transduction mechanisms in the lens will open the possibility of pharmacological intervention in situations involving abnormal proliferation or differentiation of lens cells.
Although the specific signaling pathways leading to lens fiber cell differentiation are not yet understood, it is clear that these signals ultimately result in cell cycle withdrawal and changes in gene expression. Several lines of investigation indicate that the active, hypophosphorylated form of the tumor suppressor protein pRb plays a crucial role in this process. Interestingly, however, the expression of Cdk4, the enzyme primarily responsible for phosphorylating pRb and initiating entry into S phase, is not downregulated during normal fiber cell differentiation. Both Cdk4 and its regulatory protein cyclin D are expressed at high levels during fiber cell differentiation. However, activity of the Cdk4/cyclin D complex is suppressed by the Cdk inhibitor p57kip2, thus preventing pRb phosphorylation and leading to cell cycle arrest. The central role of this Cdk inhibitor in normal fiber cell differentiation has recently been demonstrated by homozygous disruption of the p57kip2 gene.
In addition to cyclin D/Cdk4, several other cyclins and Cdks are expressed in differentiating lens fiber cells, including p35/Cdk5 and, in the embryonic lens, cyclin B/Cdc2. Since these kinases have also been implicated in apoptosis of certain cell types, their presence in lens fibers raises the possibility that they may be involved in the apoptotic-like events that characterize terminal differentiation of lens fiber cells. Other proteins involved in apoptosis may play roles in terminal differentiation.
Among these are members of the bcl-2 family and the proteases (or capsases) related to the interleukin-1 beta-converting enzyme. Genetic and biochemical analyses of these and other proteins that regulate cell cycle progression and apoptosis are needed to provide insight into specific genetic defects that lead to aberrant differentiation, inappropriate apoptosis, and cataract. This analysis can also provide an understanding of the uncontrolled lens cell epithelial growth characteristic of secondary cataract.
Strategic Research Questions
What are the growth factors and matrix components that regulate growth and differentiation of the lens in vivo? Is growth factor stimulation required throughout the differentiation process or only to initiate the differentiation cascade? Defining individual components involved in growth and differentiation signaling and the mechanistic details of the signaling process is a first step in identifying pathways to target in developing preventative strategies for posterior subcapsular cataract.
Which signal transduction pathways are selectively activated by signals for proliferation or differentiation? Which transcription factors are targeted by the signal transduction pathways? Identifying signaling pathways and transcription factors targeted by these pathways are crucial to understanding differentiation and cell cycle regulation in the lens. Strategies involving pharmacological agents that target signal transduction pathways should provide information about the relevant signal transduction mechanisms in the lens and open the possibility of pharmacological intervention in situations involving abnormal proliferation or differentiation of lens cells.
What regulates expression of the cyclin-dependent kinase inhibitor p57kip2? What target genes other than p57kip2 need to be turned on or off to initiate differentiation? A critical step in cell cycle regulation requires the suppression of cell cycle activating proteins. To acquire a complete picture of lens cell growth and differentiation, the details of this step in cell cycle regulation need to be elucidated.
What are the roles of cyclins, Cdks, and other cell cycle regulatory proteins during fiber cell differentiation? Several cyclins and Cdks are expressed in differentiating lens fiber cells. Transgenic and knockout technology should enable investigators to sort out the specific roles for these proteins.
Are apoptosis and terminal differentiation of fiber cells related processes? Genetic and biochemical analyses of proteins that regulate cell cycle progression and apoptosis may provide insight into specific genetic defects that lead to aberrant differentiation, inappropriate cell death, and cataract, as well as an understanding of the uncontrolled lens cell epithelial growth characteristic of secondary cataract.
Objective 7: Characterize, at the molecular level, the ion channels, transporters, and gap junction proteins needed to maintain lens homeostasis; determine what roles perturbations in these systems play in cataract formation.
Research Needs and Opportunities To perform its refractory function, the lens must be transparent; hence, light-scattering elements are precluded. Consequently, the lens is an avascular tissue that comprises precisely packed multiple layers of cells. Because of the absence of blood vessels, the lens relies on the epithelial cells to take up solutes and ions from the aqueous humor. The epithelial cells then must provide all the necessary metabolic requirements to cells in the lens. To accommodate its unique situation, the lens uses a vast array of channels, pumps, and intercellular connections at gap junctions to distribute nutrients, remove metabolites, and maintain the proper ionic balance for both epithelial and lens fiber cells.
The regional distribution of sodium/potassium pumps, channels, and gap junctions throughout the lens likely creates an internal circulatory system that compensates for the lack of a vasculature system. A system in which ions and water enter the lens along intercellular clefts, cross fiber cell membranes, flow from cell to cell through gap junction channels toward the surface, and cross surface cell membranes to complete the loop has been postulated. The driving force behind the direction of these fluxes is a difference in electromotive potential between outer epithelial cells and inner fiber cells and the distribution of gap junctions in differentiating fiber cells. Because the system of ion fluxes is critical to maintaining transparency, investigators have directed their attention to understanding the details of the various components of the system.
For many years, understanding the molecular mechanisms involved in gap junction function was hampered by a lack of knowledge concerning which membrane polypeptides were directly involved in channel formation. Using methods of modern molecular biology, many of these proteins (called connexins) have been identified and cloned, making it possible to ask the fundamental questions concerning gap junction function. Recently, the tools have been developed that will facilitate definitive examination of the roles and the importance of lens gap junctions. Antibody probes allow the detection of all of the lens connexins. DNA clones permit the detection of the connexin genes and mRNAs and the molecular manipulation of their expression, both in tissue culture systems and in mouse transgenic and knockout lines. Electrophysiological methods are now available for examining lens intercellular communication and studying the biophysical properties of lens gap junction channels.
The importance of gap junctions in maintaining transparency has recently been demonstrated. Experiments were conducted using mice in which deletion of the fiber-specific connexin 46 was introduced. Mice homozygous for the deletion developed nuclear opacities within 3 weeks of birth. The association of these nuclear cataracts with proteolysis of crystallins underscores the significance of gap junctions to the overall homeostasis of the lens.
A number of ion-conducting channels have been identified. Substantial diversity has been discovered in lens potassium channels and suggests that the regulation of transmembrane voltages will likely be complex. Furthermore, several of these channels have been shown by molecular biology to be identical to those from excitable tissues. Asymmetric placement of lens ion transporters has been shown to result in spatially varying standing current flow in the lens. Models of these processes support an internal circulation of fluid in the lens. Chloride channels have been shown to be involved in the lens response to nonisosmotic media. However, specific chloride channels have yet to be identified. Sodium conductance in epithelial cells appears to be via a nonselective cation channel. However, the relevance of the leak conductance of sodium by this channel has not been established. Major intrinsic protein (MIP) from lens fiber membranes has been shown to function as a water channel. Interestingly, lack of this protein occurs in an experimental line of cataractous mice.
Understanding the ion conductance in the lens potentially has clinical relevance. The formation of many kinds of cataracts is accompanied by a rise in both intracellular sodium and calcium with a concomitant loss of intracellular potassium. The molecular nature of the transporting proteins involved in these derangements is essentially unknown. It is clear that lens cell membranes, like other cell membranes, have little permeability to ions in the absence of specific transport proteins; therefore, alterations in these proteins must be involved in the ionic alterations that accompany cataract formation. A detailed structural and functional knowledge of the specific transport proteins involved in these ionic changes is needed, especially in those involved in sodium and calcium movements in the lens. Such movements can occur by adenosine triphosphate (ATP) driven pumps, specific ionic channels, nonspecific ionic channels, and nonchannel-based transporters and can be influenced by transmembrane voltages in the lens. Thus, chloride channels, potassium channels, and electrogenic transporters involved in maintaining lens voltages are critical for controlling lens sodium and calcium movements. It is, therefore, just as important to understand the molecular nature of these transporters as it is to understand those for sodium and calcium movements. Recent advances in molecular biology, electrophysiology, and cellular imaging now allow the molecular nature of these processes to be investigated.
Lastly, the mechanisms that regulate transport need to be understood. Muscarinic and purinergic receptors have been found in lens epithelial cells. Both are involved in intracellular calcium regulation. The signal pathways that are linked to muscarinic and catecholamine receptors, purinoceptors, and other receptors remain to be characterized. As channels are identified and characterized, there will be new opportunities to address questions about their localization, functional integration, and regulation.
Strategic Research Questions
What is the importance of each of the connexins in maintaining lens homeostasis? What is the contribution of each of the connexins to the maintenance of lens transparency? The opportunity to explore the role of gap junctions in lens in maintaining lens homeostasis and transparency has been made possible by the cloning of connexin proteins. With tools such as antibody probes and cloned genes for transfections, experimental strategies to perform in situ histochemical and electrophysiological analyses should provide insight into the function of gap junctions in the lens. Transgenic and knockout technology is proving to be a valuable tool in ascertaining the role of connexins in maintaining lens transparency.
What are the essential molecules that pass through the lens gap junctions, and how is their passage controlled? Identifying the molecules transported by gap junctions and how their passage is regulated will enhance understanding lens physiology. This information should provide significant insights into how changes in levels of essential small molecules, such as glutathione and osmolytes, contribute to age-related and diabetic cataract, respectively.
What is the role of gap junctions in lens development? What molecular mechanisms control expression of connexin genes during lens development? With the availability of cloned genes, connexin-encoding genes can be introduced or deleted in developing embryos vis-à-vis transgenic and knockout technology in mice and retroviral technology in the chick. The ability to genetically alter expression of connexins in model systems will allow investigators to pursue strategies to study the role and regulation of connexins in development.
What metabolic factors control opening and closing of the gap junction channels? How do posttranslational modifications and proteolysis contribute to the regulation of gap junctions? Data suggest that posttranslational modifications of connexins regulate gating properties and assembly of gap junctions. Identifying and characterizing posttranslational modifications in gap junctions will expand science's database of knowledge on the role of posttranslational modifications in the maintenance of lens homeostasis.
How are the subunits of gap junctional proteins assembled into functional channels and plaques? How connexins are assembled into functional channels across fiber-fiber, epithelial-epithelial, and fiber-epithelial cell types is unknown. Strategies that combine the tools of cell and molecular biology should be useful in delineating the critical steps in assembly.
What regulatory signaling pathways exist and how do they alter channel function? Identifying the regulatory pathways that control channel function is critical to understanding how the lens' internal circulatory system functions to maintain lens health.
What are the structural and detailed biophysical properties of each ion channel and pump in lens cells? The lens cells likely contain a complex array of ion channels and pumps in the lens internal circulatory system. Characterization of each individual component is necessary to develop a complete picture of this circulatory system.
How are the channels and pumps targeted to the plasma membrane or to organelle membranes and how is their distribution between epithelium and fibers regulated? Membrane targeting creates an asymmetric distribution of transporters. Presumably this structural distribution reflects a functional specialization that contributes to driving the circulation of ions and fluids. Characterizing membrane targeting of transporters and the distribution of transporters throughout the lens are essential to understanding the driving forces behind the lens circulatory system.
How do changes in ion fluxes apply to cataract models and human cataract studies? Because the formation of many kinds of cataracts is accompanied by a rise in both intracellular sodium and calcium with a concomitant loss of intracellular potassium, strategies to identify the molecular nature of the transporting proteins involved in these derangements are needed. An understanding of ionic changes that accompany cataract formation will require a structural and functional knowledge of the specific transport proteins involved, particularly those subserving sodium and calcium movements in the lens.
Objective 8: Define the mechanisms that regulate the cellular and subcellular architecture of the lens, with special emphasis on the contribution of minor constituents and their progressive modification during aging and opacification.
Research Needs and Opportunities
While cytoskeletal proteins and membrane proteins are minor constituents of lens cells, lens-specific intermediate filaments CP49/phakinin and CP115/filensin and membrane proteins MIP and MP20 may be required for the unique organization of epithelial cells, transparent lens fibers, and lens elasticity. The molecular interactions among cytoskeleton, α-crystallins, and lens cell membranes appear to be modulated by common metabolites (ATP, glutathione, calcium, taurine, and endogenous lens peptides), which influence the symmetric differentiation of lens fibers and the normal development of the transparent lens. Variations in lens metabolites may be a response to regulators of differentiation and growth that are responsible for developing symmetric transparent lenses.
Little is known about the interactions between epithelial and fiber cells that contribute to the symmetry of developing transparent lenses. In lens cells, the preferential expression of CP49/phakinin, CP115/filensin, MIP, and MP20 may be a response to growth factors, signaling pathways, and intercellular communication involved with the regulation of lens cell differentiation that specify normal proliferation and differentiation of lens fiber cells. Defective regulation of growth and differentiation observed in transgenic mice with altered growth factor responses and knockout mice in which Rb is deleted result in asymmetric cellular organization and opacification of lenses. These mice provide an opportunity to investigate not only the contribution of the cytoskeleton and membrane proteins to lens transparency but also the relationship between cytoskeleton integrity, the cytoarchitecture, and cell signaling pathways needed to maintain transparency.
During normal lens growth and development, symmetric shells of fiber cells meet end-to-end along well-defined planes known as lens sutures. Even subtle asymmetry in suture development may be an indicator of potential defects in the cytoarchitecture. Methodology to study lens organization at the tissue and cellular levels is available. Quantitative analyses are needed to characterize the 3-D organization of lens sutures in normal and abnormal lenses. The use of confocal and other novel microscopic methods in systematic and quantitative analyses of lens cell and tissue structure will enhance researchers' understanding of the cellular organization within the lens tissue and the role of the cytoskeleton and other structural proteins in this organization. Transgenic and knockout mice with altered cytoarchitecture provide an excellent model system for relating structural studies of sutures to the cell biology of the cytoskeleton and the signaling pathways that regulate it. Finally, all such studies must lead to a better understanding of transparency in the human lens. Ultimately, human tissue must be analyzed in order to relate findings in model systems to the processes of aging and cataract formation.
Strategic Research Questions
How is the expression of lens-specific cytoskeletal and membrane components regulated during normal development or during loss of transparency in model systems and human lenses? Aberrations in subcellular architecture may arise as epithelial cells differentiate. Therefore, it is likely that the rearrangements of subcellular structure that occur as epithelial cells differentiate into fiber cells and fiber cells mature are regulated. Transgenic experiments suggest that defective regulation of growth and differentiation is accompanied by defects in cell organization. Technology to probe for differential expression of cytoskeletal and membrane components during normal and defective fiber cell differentiation and maturation is available in model systems. Strategies need to be developed to transfer information from model systems to humans.
Are progressive modifications of the cytoskeleton and cytoarchitecture of human epithelial cells during aging and cataract formation a response to molecular events involving the abnormal expression of growth factors, receptor distribution, and/or signaling functions? Experiments in transgenic mice suggest that defective regulation of growth and differentiation observed in transgenic mice with altered growth factors result in asymmetric cellular organization and opacification of lenses. These mice provide an opportunity to investigate the relationship between cytoskeleton integrity, cytoarchitecture, and cell-signaling pathways.
What changes in suture pattern occur during human cataract formation, normal lens aging, and/or changes in elasticity? Identifying changes in suture pattern during cataract formation and aging will provide structural information at the tissue level that may suggest fundamental underlying changes occurring at the cellular level.
How does the progressive modification of lens epithelial cells as observed in vivo result in either quantitative differences in 3-D organization or transparency of lens fiber cells in normal and opaque human lenses? Quantitative analyses are needed to characterize the 3-D organization of lens sutures in normal and abnormal lenses. The use of confocal and other novel microscopic methods in the analyses of lens cell and tissue structure will enhance understanding of the cellular organization within the lens tissue.
Will knockout or overexpression of lens-specific cytoskeletal and membrane components result in decreased or improved lens transparency and elasticity? This technology will enable scientists to answer fundamental questions concerning the contribution that the cytoskeleton and membrane proteins make to the overall functioning of the lens.
Objective 9: Understand the basis of lens accommodation and presbyopia at the molecular and mechanistic levels.
Research Needs and Opportunities
The ability to accommodate progressively decreases with age, eventually requiring many individuals in their midforties to wear reading glasses. This inability to accommodate adequately is termed presbyopia. Presbyopia affects everyone and has enormous economic impact as well as a disconcerting and frustrating impact on quality of life. Consider the fact that in 1997 there were approximately 93.6 million people over the age of 45 in the United States. First, there is the economic cost of increasingly poor work performance from a person's denial of the need for a reading aid. Second is the cost of obtaining an aid. If 25 percent of those age 45 and older received an eye examination (approximately $60) and bought inexpensive bifocals (about $150), the economic cost would be $4.9 billion (ignoring transportation costs and lost time from work). If another 25 percent solved their near-reading problem by buying nonprescription drugstore readers for $20, the additional cost would be $468 million. The economic and quality-of-life improvements of identifying preventive measures or interventions that delay or prevent both the loss of accommodation and the onset of presbyopia are obvious and would be of benefit to everyone, particularly when considering that these may be an individual's most economically productive and influential years.
The exact reasons that the accommodative system fails remain elusive. It has long been suspected that as the lens ages it becomes thicker and less pliable, losing its ability to change shape and consequently its ability to accommodate. There is also some evidence to suggest that the muscle controlling the tension of the zonular attachments to the lens capsule may play a significant if not key role in the failure of the accommodative mechanism to respond adequately with increasing age. In addition, there is preliminary evidence that near-vision can actually be restored by physically changing the relationship between the muscle and lens. Lastly, the role of extralenticular elastic tissues in the ciliary muscle and choroid, in pathophysiology and as therapeutic targets, needs to be explored.
In a somewhat different but related subject, it is known that as the lens ages, the anterior posterior thickness increases and the equatorial diameter decreases, leading to the paradox that a bigger, thicker lens is optically less powerful, as opposed to being more powerful.
In younger adults, malfunctioning of the accommodation mechanism has been implicated as a likely cause of adult-onset myopia. Differential accommodation between the two eyes is also likely to be involved in the development of anisometropia, a condition in which the two eyes have different refractive properties.
Because of recent advancements in measuring objectively the aberrations of the human eye in vivo, and because researchers have a better understanding of the biometry of the aging lens, researchers are in a position to accurately model gradient index changes within the lens that could explain the thick lens paradox. Similarly, recent advances in the resolution of a variety of noninvasive measuring techniques like high-frequency ultrasound should resolve the geometric uncertainties of the relationships between the ciliary muscle, zonules, capsule, and lens during accommodation and as a function of age. These advances suggest ways to delay or prevent presbyopia.
Strategic Research Questions
How do the physical relationships between the ciliary muscle, zonules, capsule, and lens change with accommodation and as a function of age? The resolution of a variety of noninvasive measuring techniques has advanced to the point that they can be used to resolve the relationships between the ciliary muscle, zonules, capsule, and lens. Incorporating these techniques into population-based studies would clarify the role of these issues as they relate to accommodation and age.
Can medical therapy (e.g., antioxidants, lens growth inhibitors) favorably alter the accommodation versus age function? Scientists need to explore innovative approaches to treatment.
What is the role of accommodation in the onset of adult myopia? The etiology of this condition needs to be defined. Studies suggesting that malfunctioning of the accommodation mechanism underlies adult-onset myopia requires confirmation.
LENS AND CATARACT PANEL
CHAIRPERSON
Larry Takemoto, Ph.D.
Kansas State University
Manhattan, KS
PANEL MEMBERS
Usha Andley, Ph.D.
Washington University Eye Center
St. Louis, MO
Ray Applegate, O.D., Ph.D.
University of Texas Health Science Center
San Antonio, TX
Eric C. Beyer, M.D., Ph.D.
Washington University School of Medicine
St. Louis, MO
John Clark, Ph.D.
University of Washington School of Medicine
Seattle, WA
Donita Garland, Ph.D.
National Eye Institute, NIH
Bethesda, MD
James Goldman, M.D., Ph.D.
Columbia University
New York, NY
Robert Grainger, Ph.D.
University of Virginia
Charlottesville, VA
Joseph Horwitz, Ph.D.
University of California at Los Angeles
Los Angeles, CA
Marjorie F. Lou, Ph.D.
University of Nebraska - Lincoln
Lincoln, NE
Paul Overbeek, Ph.D.
Baylor College of Medicine
Houston, TX
James Rae, Ph.D.
Mayo Clinic
Rochester, MN
Robert Sperduto, M.D.
National Eye Institute, NIH
Bethesda, MD
Dwight Stambolian, M.D., Ph.D.
University of Pennsylvania School of Medicine
Philadelphia, PA
Peggy Zelenka, Ph.D.
National Eye Institute, NIH
Bethesda, MD
NEI STAFF
Ellen S. Liberman, Ph.D.
National Eye Institute, NIH
Bethesda, MD


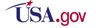